Explosive Nucleosynthesis
Nucleosynthesis is the creation of new, and heavier, elements from precursor lighter elements. To take an example, our Sun presently converts hydrogen to 4He nuclei by way of a series of nuclear reactions called the proton-proton chains. When it has exhausted the hydrogen in its core, having converted it all to 4He, the 4He will become the next nuclear fuel and will be itself converted to 12C and 16O. This is "quiescent" nucleosynthesis; so-called, because the fusion reactions occur under non-explosive conditions within the core of the star.
Explosive nucleosynthesis occurs when a star, or some portion of the star, undergoes a thermonuclear runaway that results in an explosion. Supernovae are some of the most spectacular examples of this phenomenon. More common, however, are novae; these are thermonuclear runaways on the surface of a white dwarf (WD) star in a binary star system. Approximately 30 per year occur in our galaxy.The present understanding of these systems is that hydrogen-rich material from the binary companion star is transferred to the WD, forming a hot, dense layer, laden with nuclear fuel, enveloping the WD surface. Under the extreme pressure and temperature of this environment, conditions are met wherein protons (the hydrogen in the envelope) begin to fuse with the nuclei comprising the composition of the WD surface. These nuclei forming the WD surface composition are called "seed nuclei". In the case where the WD is of the "oxygen-neon"-type, the seed nuclei are predominantly 16O and 20Ne. Starting with proton captures onto these seeds, a nuclear process is started wherein heavier nuclei are synthesized by means of the competition between the rates of these successive proton captures onto short-lived radioactive nuclei and the concommittant β-decay rates of these nuclei. The nuclear energy released in this process leads to a thermonuclear runaway within the envelope resulting in a thermonuclear runaway that causes the ejection of the envolope and the newly synthesized nuclei into space. A Hubble photograph of a candidate oxygen-neon nova is shown in the image above; one can see the central WD surrounded by a clumpy ejecta of material, blasted off the WD surface by the thermonuclear runaway.
Observationally, oxygen-neon novae are known to produce elements up to calcium, 40Ca. This means that all nuclei between 20Ne and 40Ca are newly synthesized by the proton capture reactions mentioned above. Almost all of the radioactive nuclei produced in this process are sufficiently short-lived that they have already β-decayed to their stable isobars long before the ejecta phase seen in the photo above. However, there are several of these nuclei that are sufficiently long-lived that they could be present in the ejecta phase. These are 22Na (t1/2 = 2.6 yr), 26Al (t1/2 = 7.17 × 105 yr) and 34Cl (t1/2 = 32 min). All 3 of these nuclei β-decay. These decays populate excited nuclear states in the daughter nuclei, which then decay by gamma-ray emission. These gamma rays (1.28 MeV from 22Na decay, 1.81 MeV from 26Al decay, 2.13 MeV from 34Cl decay) are, in principle, observable by past and present orbiting gamma-ray observatories. Any detection of these gamma-rays would provide direct window into the nuclear processes occurring within the thermonuclear runaway of these events. Indeed, an observation of these gamma rays could even provide information on the temperatures acheived in these explosions. At present, there is only one indication of gamma-ray emission from 22Na decay [A. Iyudin, Astron. Rep. 54, 611 (2010); doi: 10.1134/S106377291007005X], and it comes from a carbon-oxygen nova, which themselves are not expected to produce significant amounts of these gamma-ray isotopes. None of these gamma-rays have even been observed from nova candidates that are expected to produce them. This situation, thus, remains a puzzle in nuclear astrophysics, and it is not clear if the problem resides in our understanding of the physics of the explosion mechanisms or in the underlying nuclear physics inputs used in the explosion models.
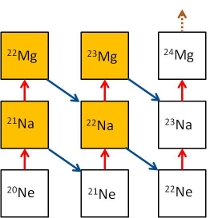
As an illustrative example of how the gamma-ray isotopes are produced, the figure to the left shows how the gamma-ray emitter 22Na is produced. Red arrows denote proton captures, blue arrows denote β-decays. Starting with proton capture on the seed 20Ne, 22Na can be produced by either proton capture onto 21Na to 22Mg followed by β-decay. Two reaction pathways can produce 22Na after proton capture onto 20Ne: proton capture onto 21Na to 22Mg, followed by β-decay to 22Na; β-decay from 21Na followed by proton capture onto 21Ne. Furthermore, 22Na is itself destroyed by proton capture to 23Mg. The final abundance, then, of 22Na in the ejecta and, hence, the amount of 1.28 MeV gamma-rays we can expect to observe in our orbiting gamma-ray telescopes, is sensitive to the rates of these reactions. Until recently, the 21Na(p,γ)22Mg reaction rate had never been measured; it requires a high intensity beam of short-lived radioactive 21Na nuclei which, until the advent of new radioactive ion beam facilities such as ISAC, could not be produced. The result of the direct measurement of the 21Na(p,γ)22Mg reaction rate [S. Bishop et al., Phys. Rev. Lett. 90, 162501 (2003); doi: 10.1103/PhysRevLett.90.162501 and J. D'Auria et al., Phys. Rev. C 69, 065803 (2004); doi: 10.1103/PhysRevC.69.065803], combined with nova nucleosynthesis models, indicated an overall reduction in the expected amount of 22Na as compared to previous estimates. In a similar manner as seen in the figure, the production and destruction pathways for the 26Al and 34Cl gamma-ray emitters have some proton capture reaction rates that are also entirely unmeasured.
As the above figure shows, the reaction flow proceeds along a pathway that is only 1 or 2 neutrons removed from the nearest stable isotopes. The proton capture reaction rates in novae explosions depend on certain nuclear properties of the nuclei involved: excitation energies, spins, and lifetimes of the excited states. And because the reaction flow is only 1 or 2 neutrons removed from stability, these important properties for many of these nuclei can be measured using indirect nuclear reaction techniques at tandem accelerator laboratories, such as the Maier-Leibnitz laboratory here at TUM.
We have completed construction of a new experimental facility that will measure the lifetimes of excited nuclear states in nuclei involved in nova explosions; and the existing high resolution momentum spectrometer allows us to map out the existence and corresponding energies of excited states in those nuclei involved in these explosions.